A, B, Cs of Fusion Power.
I have repeated stated that fusion power is the ultimate answer to Global Warming (GW). This week I’m going to give you a primer and a status report on fusion power. Nuclear Fusion is probably one of the most important physical processes in the universe. Fusion created the building blocks of life from the most basic constituents of the early universe. While the energy we humans use today has been transformed into various other forms, locked up in chemical bonds of the carbohydrates in our food and hydrocarbons that power our cars, it originally fell to earth as solar energy from our closest neighboring fusion reactor: the Sun. The prospect of controlled fusion has captivated generations, but other than the hydrogen bomb, we have not been able to successfully build a fusion device that generates more energy than we put into it. The promise of fusion powerplants is one of truly unlimited energy production, largely free of environmental concerns, and without legacy radioactivity for future generations. When we are able to thoroughly control a fusion reaction here on earth, we will have paved the way for the human race to thrive long into the future.
To begin let's take examine what fusion is, and how it works...
In our Sun, hydrogen is fused into helium, and when the hydrogen runs out, helium can be fused into carbon. When the carbon runs out, elements such as Neon, Oxygen, and Silicon, can be burned, all the way up to radioactive Nickel which decays into Iron-56 lying at the crest of the curve of Binding Energy (figure 1 below).
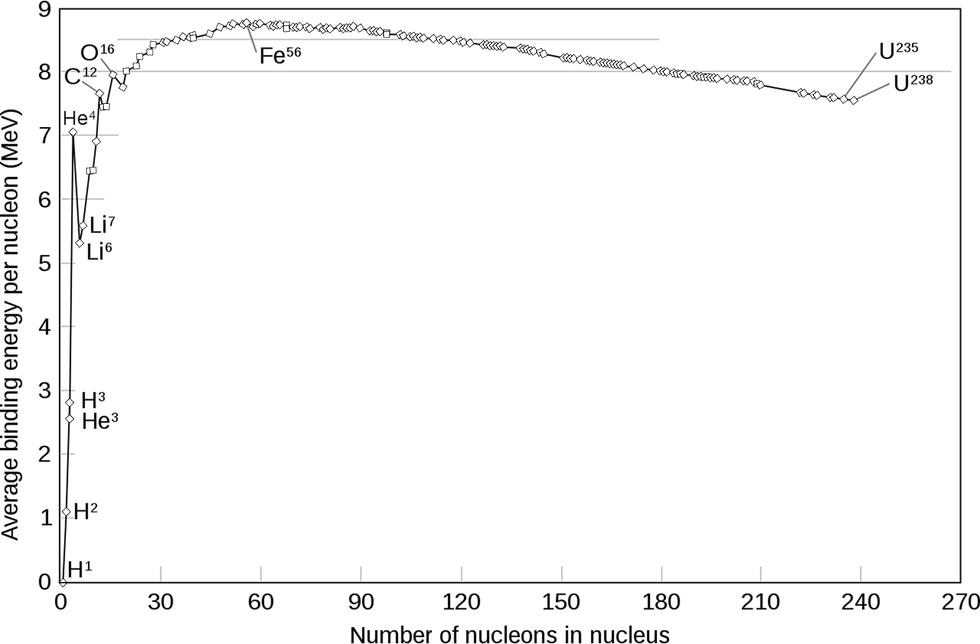
Figure 1 - Atomic Binding Energy Curve
Binding energy is the energy required to disassemble the nucleon into its component parts. As hydrogen and Helium atoms fuse into heavier atoms in the sun the binding energy increases, and the resulting products have more energy than the original atoms. This keeps the sun shining and the Earth warm. This initial fusion of hydrogen and helium in the sun is what we are trying to duplicate in fusion reactors on Earth. Eventually the fusion reaction in the sun will reach Iron-56 which is at the peak of the binding energy curve and is the most stable atom known. Once everything below Iron is gone, the sun collapses into a dwarf star.
To the right of Iron, the Binding Energy decreases again and hence these atoms are less stable. Therefore, the atoms to the right can be fissioned to release net energy in their daughter atom products. The best example of this is Uranium-235. Note that elements beyond Iron-56 are created during supernovas of stars much heavier than our sun. Once a high mass star begins producing Iron, it has only a fleeting life left. Without being able to produce energy from fusion it will lose the outward pressure keeping it intact. It will soon implode, and the resulting energy release, (Supernova) generates the elements heavier than Iron and enriches deep space with many of the periodic table's most diverse elements, including the heaviest ones like Uranium. Actually, elements heavier than Uranium are produced in Supernovas, but their half-lives are all so short that they have all decayed away on Earth.
Now that you understand the basics of the fusion and fission processes, we can get into the details of what it takes to make them happen. Some atoms are easily fissionable according to the makeup of their nucleus, for instance U-235. Others like U-238 are not fissionable unless they gain a proton and become Plutonium-239. It is relatively easy to assemble a fission reactor or an atomic bomb, but that is beyond the scope of this article.
Getting controlled fusion to happen is much more difficult which is why it has been twenty to thirty years away for my entire lifetime. A substantial energy barrier of electrostatic forces must be overcome before fusion can occur. At large distances, two naked nuclei repel one another because of the repulsive electrostatic force between their positively charged protons. If two nuclei can be brought close enough together, however, the electrostatic repulsion can be overcome by the quantum effect in which nuclei can tunnel through coulomb forces. This effect is shown in figure 2 below.
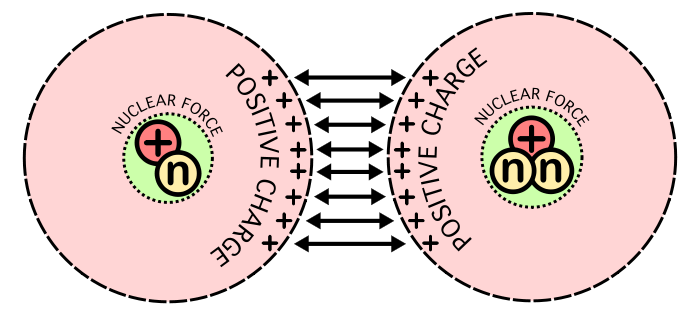
Figure 2 - The electrostatic force between the positively charged nuclei is repulsive, but when the separation is small enough, the quantum effect will tunnel through the wall. Therefore, the prerequisite for fusion is that the two nuclei be brought close enough together for a long enough time for quantum tunnelling to act.
The reacting atoms need enough kinetic energy to overcome the coulomb barrier, and this is measured as temperature of the plasma. The average fusion collision cross-sections for candidate fusion fuels versus the plasma temperatures in KeV are plotted in figure 3 below.
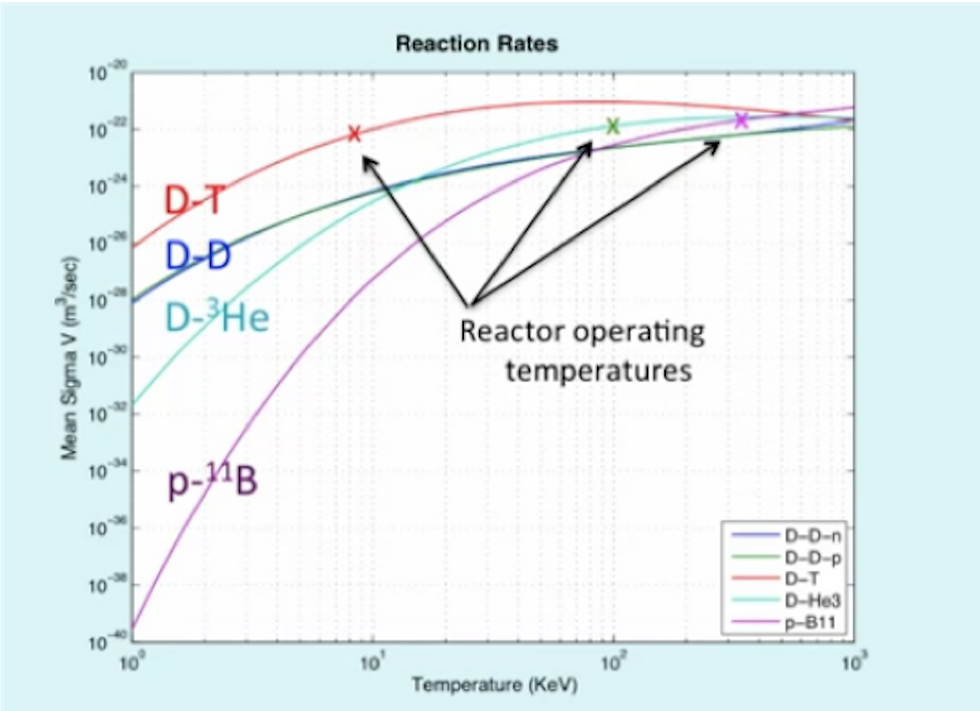
Figure 3 - Fusion Cross-sections vs Temperature
The fusion reaction rate in any fusion reactor is multiplicative total of the number density of atoms in the reacting plasma times the fusion cross section (σ) which is a measure of the probability of a fusion reaction as a function of the relative velocity of the two reactant nuclei. If the reactants have a distribution of velocities, e.g., a thermal distribution, then it is useful to perform an average over the distributions of the product of cross-section and velocity. This average is called the 'reactivity', denoted <σv>. The reaction rate (fusions per volume per time) is <σv> times the product of the reactant number densities:
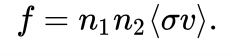
If a species of nuclei is reacting with a nucleus like itself, such as the DD reaction, then the product n1 n2 must be replaced by n2/2.
<σv> increases from virtually zero at room temperatures up to meaningful magnitudes at temperatures of 10-100 keV. At these temperatures, well above typical ionization energies (13.6 eV in the hydrogen case), all the fusion reactants exist in a plasma state.
The fusion reaction rate times the reactor confinement time gives us the power generated per volume and if this is generated than the energy we input to reach the operating conditions, we have a net energy gain and a successful fusion reactor. The importance of confinement time is found by considering the Lawson criterion. The Lawson criterion is a figure of used in nuclear fusion research. It compares the rate of energy being generated by fusion reactions within the fusion fuel to the rate of energy losses to the environment. When the rate of production is higher than the rate of loss, and enough of that energy is captured by the system, the system is said to be ignited.
Derived in 1955, the Lawson criterion specifies the conditions that must be met for fusion to produce a net energy output (1 keV × 12 million K). From this, a fusion “triple product” can be derived, which is defined as the product of the plasma ion density, ion temperature and energy confinement time. This product must be greater than about 6×1021 keV·m−3·s for a deuterium–tritium plasma to ignite. The Lawson Criterion is shown for various Magnetic Confinement Fusion Test Reactors in figure 4 below.

Figure 4 - Lawson criterion of important magnetic confinement fusion experiments
Due to the radioactivity associated with tritium, today’s research tokamaks generally operate with deuterium only (solid dots). The large tokamaks JET(EU) and TFTR(US), however, have used a deuterium–tritium mix (open dots). The rate of increase in tokamak performance has outstripped that of Moore’s law for the miniaturization of silicon chips (Pitts et al. 2006). Many international projects (their names are given by acronyms in the figure) have contributed to the development of fusion plasma parameters and the progress in fusion research which serves as the basis for the ITER design. Note that QDT is the ratio of the net power generated during a pulse to the power input and must be significantly better than 1 to have a successful design. I’ve decided to cover only Magnetic Confinement Fusion Reactors this week. I’ll expand this to cover Inertial Confinement and Field Reversed Magnetic Confinement next week.
Magnetic Confinement Fusion (MCF) has been pursued for about 50 years and works by heating a dilute plasma (1014 ions per cm3) to high temperatures, around 20 keV (~200 million C), confined by a toroidal magnetic field. For reference, air at sea level is about 1019 atoms per cm3, so the conditions in the reactor are nearly a hard vacuum. The current “prototype MCF” is the International Thermonuclear Experimental Reactor (ITER) Tokamak which is 12.4 m in diameter, has an on axis magnetic field of 5.3 T, and weighs 23,000 tons. It is currently being upgraded to test the magnetic approach with pulse lengths up to 20 minutes. This has proven to be extremely challenging, which explains why fusion research has taken many years to reach the current advanced technical state. Magnetic confinement experiments have uncovered numerous plasma instabilities which have systematically been overcome to reach the levels shown in current test reactors. I will cover those best-in-class reactors below to show current Fusion progress. A cutaway of the ITER is shown in figure 5 below. It is currently scheduled to go operational in 2035 and generate 500 MW of power during a 1000 second pulse.
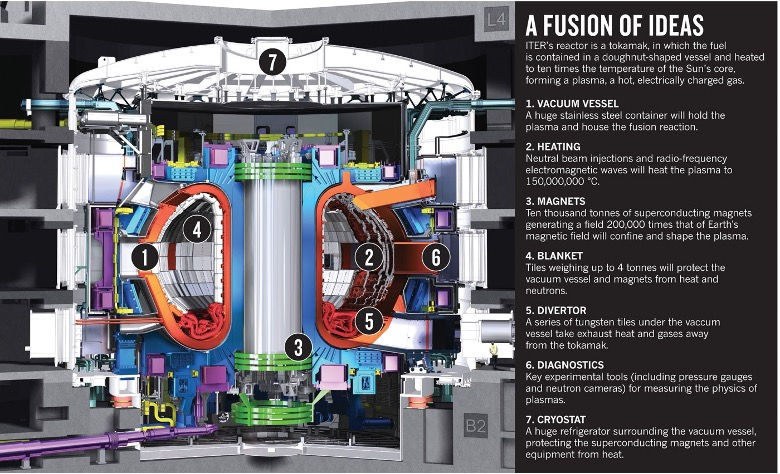
Figure 5 - International Thermonuclear Experimental Reactor (ITER)
The ITER needed to be huge because of its superconductors. They could only support limited magnetic field strengths (B-fields) which controls the plasmas gyration radii and this drove the size up. Modern superconductors can support much higher B-Fields before they quench, and this has allowed current MCF test reactors to be much smaller (~ 3.7 m in diameter) and cheaper.
SPARC Tokamak
SPARC is a tokamak under development by Commonwealth Fusion Systems (CFS) in collaboration with the Massachusetts Institute of Technology (MIT) Plasma Science and Fusion Center (PSFC). SPARC plans to verify the technology and physics required to build a power plant based on the ARC fusion power plant concept. The ARC fusion reactor (affordable, robust, compact) is a theoretical design for a compact fusion reactor developed by MIT and PSFC. The ARC design aims to achieve an engineering breakeven of three (to produce three times the electricity required to operate the machine) while being about half the diameter of the ITER reactor and cheaper to build.
The ARC has a conventional D-shaped advanced tokamak layout, as opposed to other small designs like the spherical tokamak. The ARC design improves on other tokamaks through the use of rare-earth barium copper oxide (REBCO) high-temperature superconductor magnets in place of copper wiring or conventional low-temperature superconductors. These magnets can be run at much higher field strengths, 11.2 T on axis, roughly doubling the magnetic field on the plasma axis. The confinement time for a particle in plasma varies with the square of the linear size, and power density varies with the fourth power of the magnetic field, so doubling the magnetic field offers the performance of a machine 4 times larger. The smaller size reduces construction costs, although this is offset to some degree by the expense of the REBCO magnets. A cutaway view of the proposed SPARC Reactor is shown in figure 6 below.
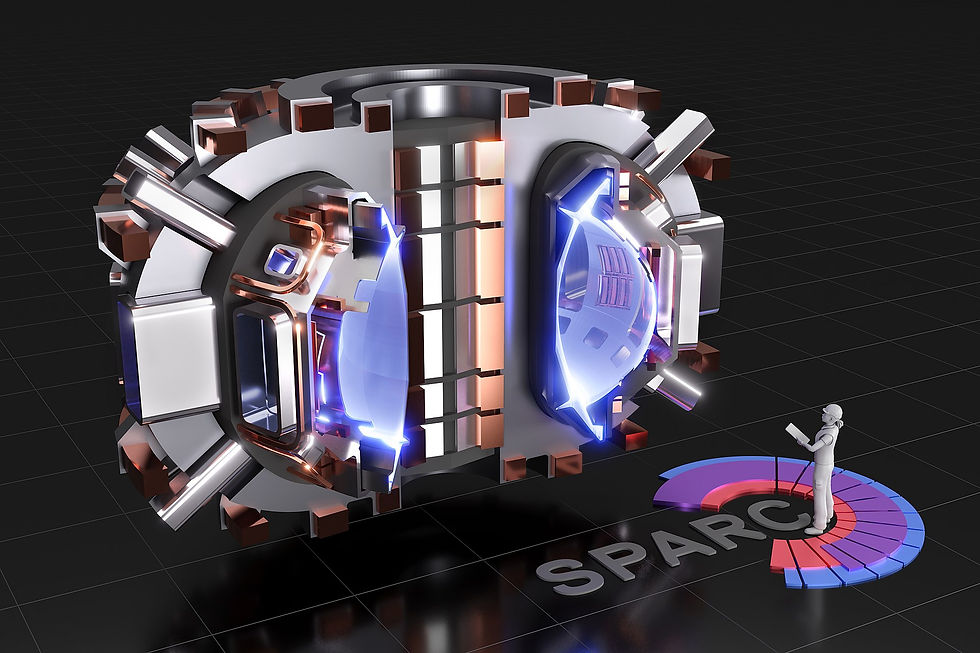
Figure 6 – Proposed SPARC Fusion Reactor (25 MW Heating & 140 MW Output)
The use of REBCO also raises the possibility of allowing the magnet windings to be flexible when the machine is not operational. This offers the significant advantage that they can be "folded open" to allow access to the interior of the machine. Accomplishing this would greatly lower the cost of maintenance, which with other designs generally requires the maintenance to be carried out through small access ports using remote manipulators. If realized, this could improve the reactor's capacity factor, an important metric in power generation costs.
SPARC is designed to achieve this with margin and may be capable of achieving up to 140 MW of fusion power for 10 second bursts despite its relatively compact size.
As of September 2020, CFS stated that they expected construction of the tokamak to begin in 2021 and take four years to complete. They expected to complete construction of the first magnet in June 2021. The advanced magnets are the key to this design and probably the prime development risk. I’ll continue to check on magnet progress. They plan to be operational in 2025.
In March 2021, CFS announced that it planned to build SPARC at its campus in Devens, Massachusetts.[10]
The Experimental Advanced Superconducting Tokamak (EAST)
EAST with the internal designation HT-7U (Hefei Tokamak 7 Upgrade), is an experimental superconducting tokamak magnetic fusion energy reactor in Hefei, China. The Hefei Institutes of Physical Science is conducting the experiment for the Chinese Academy of Sciences. It has operated since 2006.
It is the first Chinese tokamak to employ superconducting toroidal and poloidal magnets. It aims for plasma pulses of up to 1,000 seconds. It is shown in Figure 7 below.
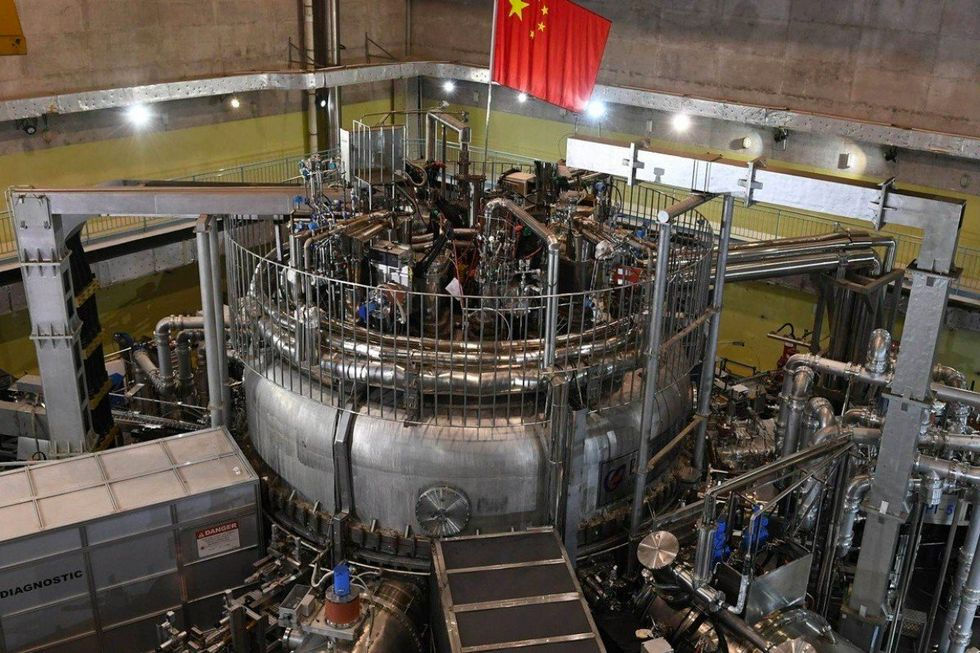
Figure 7 - EAST (Experimental Advanced Superconducting Tokamak or HT-7U)
EAST followed China's first superconducting tokamak device, dubbed HT-7, built by the Institute of Plasma Physics in partnership with Russia in the early 1990s.
The project was proposed in 1996 and approved in 1998. According to a 2003 schedule,[1] buildings and site facilities were to be constructed by 2003. Tokamak assembly was to take place from 2003 through 2005.
Construction was completed in March 2006 and on September 28, 2006, "first plasma" was achieved.
According to official reports, the project's budget is CNY ¥300 million (approximately US$37 million), some 1/15 to 1/20 the cost of a comparable reactor built in other countries.[2]
Recent History - Phase I
On September 28, 2006, first plasma was achieved—the first test lasted nearly three seconds, and generated an electrical current of 200 kiloamperes.[3]
By Jan 2007 "the reactor created a plasma lasting nearly five seconds and generating an electrical current of 500 kilo amperes".[4]
In May 2011, EAST became the first tokamak to successfully sustain H-Mode plasma for over 30 seconds at ~50 million Kelvin.
Recent History - Phase II
On May 19, 2014, after nearly 20-month-long upgrading break since September 2012, EAST was ready for the first round of experiments in 2014.
By May 2015, EAST was reporting 1 MA currents, and H-mode for 6.4 seconds.
In February, 2016, a plasma pulse was maintained for a record 102 seconds at ~50 million °C. Plasma current of 400kA and a density of about 2.4 x 1019/m3 with slowly increasing temperature.
On November 2, 2016, EAST became the first tokamak to successfully sustain H-Mode plasma for over a minute at ~50 million °C.
On July 3, 2017, EAST became the first tokamak to successfully sustain H-Mode plasma for over 100 seconds at ~50 million °C.
On November 12, 2018, EAST reached a milestone of 100 million °C electron temperature.
In May, 2021, EAST reached a milestone of 120 million °C electron temperature (10.5 keV) for 101 seconds.
Physics objectives
EAST was designed to test:
· Superconducting Niobium-titanium poloidal field magnets, making it the first tokamak with superconducting toroidal and poloidal magnets
· Non-inductive current drive
· Pulses of up to 102 seconds with 0.5 MA plasma current
· Schemes for controlling plasma instabilities through real-time diagnostics
· Materials for diverters and plasma facing components
· Operation with βN = 2 and confinement factor H89 > 2
Technical specifications - Major radius: 1.85 m (6 ft 1 in) Minor radius: 0.45 m (1 ft 6 in) Magnetic field: 3.5 T (on axis) Heating power: 7.5 MW: Discharge duration: 102 s: Plasma current: 1.0 MA
Korea Superconducting Tokamak Advanced Research (KSTAR) Fusion Reactor
The KSTAR or "superconducting nuclear fusion research device") is a magnetic fusion device at the Korea Institute of Fusion Energy in Daejeon, South Korea. It is intended to study aspects of magnetic fusion energy which will be pertinent to the ITER fusion project as part of that country's contribution to the ITER effort. The project was approved in 1995 but construction was delayed by the East Asian financial crisis which weakened the South Korean economy considerably; however the construction phase of the project was completed on September 14, 2007. The first plasma was achieved in June 2008.
KSTAR is one of the first research tokamaks in the world to feature fully superconducting magnets, which again will be of great relevance to ITER as this will also use superconducting magnets. The KSTAR magnet system consists of 16 niobium-tin direct current toroidal field magnets, 10 niobium-tin alternating current poloidal field magnets and 4 niobium-titanium alternating current poloidal field magnets. It is planned that the reactor will study plasma pulses of up to 20 seconds duration until 2011, when it will be upgraded to study pulses of up to 300 seconds duration. The upgraded reactor vessel will have a major radius of 1.8 m, a minor radius of 0.5 m, a maximum toroidal field of 3.5 Tesla, and a maximum plasma current of 2 megaampere. As with other tokamaks, heating and current drive will be initiated using neutral beam injection, ion cyclotron resonance heating (ICRH), radio frequency heating and electron cyclotron resonance heating (ECRH). Initial heating power will be 8 megawatt from neutral beam injection upgradeable to 24 MW, 6 MW from ICRH upgradeable to 12 MW, and at present undetermined heating power from ECRH and RF heating. The experiment will use both hydrogen and deuterium fuels but not the deuterium-tritium mix which will be studied in ITER.
As you can see there is a chronological progression in capability from the KSTAR to EAST and onto SPARC. EAST may be able to demonstrate breakeven power fairly soon, but I would bet that SPARC will be the first Fusion Reactor to demonstrate significant power output (~ 140 MW).
This note shows that Magnetic Confinement Fusion is only four of five years away. I will show that some other fusion concepts may be as close or closer in next week’s note.
Thanks for Reading,
Dana Andrews
retiredrocketdoc.com
Comments